published in Chapter 12, “Reactors in satellites”, in Nuclear Energy and Nuclear Weapon proliferation, ed. SIPRI (Taylor & Francis 1979)
Introduction
At present the nuclear reactors in space are power generators designed to supply the electrical needs of instruments carried aboard the spacecraft. Although nuclear reactors could also be used as energy sources for propelling spacecraft, this second class of devices will not be discussed.
The following statement has been extracted from a UN document:
The importance of nuclear power sources in, providing the energy for space programmes may be ascribed to their considerable potential advantages over other power sources. These include: self-contained operation and independence of the amount of illumination in a given region, compact construction, simplifying the orientation system and greatly reducing the energy required to keep the vehicle in its orbit; high stability in the radiation belts of the Earth; improved weight and size characteristics, beginning with certain levels of power, as well as the possibility of improving the specific mass-energy characteristics with increased power and (assured) life.1
There is little connection, if any, between the launching of satellites which bear nuclear reactors into space orbits and horizontal proliferation, that is, the increase in the number of states or groups which possess nuclear weapons. However, the capability of such satellites to assist in such tasks as military targetting could be used as an argument by the major powers for needing more nuclear weapons. In addition, such satellites contribute automatically to the qualitative arms race, although whether they do this in such a way as to increase or decrease the chance of nuclear war is debatable. Since the arms race is continuing both quantitatively and qualitatively in many directions, and there are alternatives to nuclear reactors for powering Earth satellites, this paper does not directly discuss the influence of such reactors on vertical proliferation, as this must be a small perturbation on the overall military situation.
Two nations, the Soviet Union and the United States, have been responsible for all of the nuclear fission reactors so far launched into space. The Soviet Union has launched many such reactors, while the United States has launched only one (Snap-10A), and has preferred since then to use a radioisotope as a nuclear heat source for the production of electric energy. However, the United States Department of Defense has not abandoned nuclear reactors as possibilities for future space missions requiring greatly increased electric power.2 The differences in technologies employed by the two nations are presently overshadowed by the differences in the availability of information from them.
II. The development and use of Snap devices by the USA
A summary of nuclear power systems launched spaceward by the USA for the years 1961-77 is given in table 21.1. Of the large number of launchings, only Snapshot carried a nuclear fission reactor. The other Snap devices, Transit RTG and MHW, are radioisotope packages in which the heat from radioactive disintegration is used to produce electric power.
Snap-IDA
The objectives of the Snap-10A programme were to develop, make, test and deliver to the US Air Force for night test, a 500-watt (electrical) reactor thermoelectric system having a oneyear life. The night test was intended to achieve public acceptance of nuclear reactors in space by launching, with all necessary safety approvals, a simple forerunner to larger, more complex nuclear systems. To do this, the reactor had to develop 50 kW(t) (thermal kilowatts). The reactor used was a zirconium hydride/uranium-235 type cooled by sodium-potassium liquid metal which transports the heat to the thermoelectric hot junction. The cold thermoelectric junction is cooled by radiation into space.
Snapshot was launched at 1324 hours PST (Pacific Standard Time), on 3 April 1965, being essentially non-radioactive at the time apart from the weak natural radioactivity of uranium-235. It achieved criticality at 2315 hours that evening and was operating at full power two and a half hours later. Six days later the reactor control system was deactivated by ground command, leaving the reactor in operation, subject to its own inherent control (‘negative temperature coefficient’). The reactor ran for 43 days of power operation and was prematurely shut down because of a failure of the voltage regulator in the spacecraft.
On shutdown the system contained an inventory of radioactivity of 2 x 105 curies, which will have decayed to 100 curies after 15 years (1980) and 0.1 curie after 100 years. Snapshot was placed in a 4000-year orbit before reactor start-up, so that the level of radioactivity from fission fragments will be insignificant when the satellite re-enters the atmosphere.
Table 21.1. 1961-77. Summary of space nuclear power systems launched by the USA.
System (electrical power) | Mission | Launch date | Status | |
---|---|---|---|---|
Snap-3a (2.7We) | Transit-4a | 29 Jun 1961 | Successfully achieved orbit | |
Snap-3A | Transit-4U | 15 Nov 1961 | Successfully achieved orbit | |
Snap-9A | Transit-5UN-1 | 28 Sep 1963 | Successfully achieved orbit | |
Snap-9A | Transit-5UN-2 | 5 Dee 1963 | Successfully achieved orbit | |
Snap-9A | Transit-5UN-3 | 21 Apr 1964 | Mission aborted: burned-up on re-entry | |
Snap-10A reactor | Snapshot | 1 Apr 1965 | Successfully achieved orbit: reactor now shut down | |
Snap-19B2 | Nimbus-B-I | 18 May 1968 | Mission aborted; heat source retrieved | |
Snap-19B3 | Nimbus-III | 14 Apr 1969 | Successfully achieved orbit | |
Snap-27 | Apollo-12 | 14 Nov 1969 | Successfully placed on lunar surface | |
Snap-27 | Apollo-13 | 11 Apr 1970 | Mission aborted on way to Moon. Heat source returned to South Pacific Ocean | |
Snap-27 | Apollo-14 | 31 Jan 1971 | Successfully placed on lunar surface | |
Snap-27 | Apollo-15 | 26 Jul 1971 | Successfully placed on lunar surface | |
Snap-19 | Pioneer-10 | 2 Mar 1972 | Successfully operated to Jupiter | |
Snap-27 | Apollo-16 | 16 Apr 1972 | Successfully placed on lunar surface | |
Transit-RTG | Transit | 2 Sep 1972 | Successfully achieved orbit | |
Snap-27 | Apollo-17 | 7 Dec 1972 | Successfully placed on lunar surface | |
Snap-19 | Pioneer-2 | 5 Apr 1973 | Successfully operated to Jupiter; on to Saturn | |
Snap-19 | Viking I & II | 20 Aug & 9 Sep 1975 | Successfully launched; on Mars | |
MHW | LES 8/9 | 14 Mar 1976 | Successfully achieved orbit | |
MHW | Voyager II | 20 Aug 1977 | Successfully launched | |
MHW (475We) | Voyager I | 5 Sep 1977 | Successfully launched |
All nuclear devices listed are RTGs except Snap-10A. Source: Reference 33.
Re-entry into the atmosphere — history and policy
One of the decisions which has to be made in planning satellite missions involving ultimate reentry is whether the satellite should be designed to burn up in the stratosphere or whether it should re-enter intact. Early US experience with Snap-9A in the Transit-5BN-3 night (which failed to achieve orbit) indicated complete burn-up in the stratosphere, as planned.
However, the long-term problem of contaminating the stratosphere with successive re-entries led to a decision that RTGs (radioisotope thermoelectric generator) and reactors should reenter intact. Subsequent re-entries, Nimbus B-1 and Apollo 13 (table 21.1), left the RTGs intact, as was verified by the recovery of the Nimbus RTG from the Santo Barbara channel and post-re-entry surveys of the Apollo-13 RTG which now rests in the four-mile deep Tonga ocean trench. No radioactivity was released to the atmosphere or the ocean.
Future trends in nuclear power for space vehicles
The low ratio of available electric power to the total rate of heat energy generation, in Snap10A for example, reflects two factors: (a) the narrow relative temperature interval of the thermal cycle of the reactor, which results in low thermodynamic efficiency, and (b) the low efficiency of thermoelectric devices of that period. An obvious combination of routes towards higher electrical output without creating greater radioactive containment problems is to concentrate on the improvement of efficiencies and of the overall electric power output per unit mass of the generator (figure 21.1). The current US programmes on thermoelectric generation are directed towards more efficient conversion of radioisotope heat energy in RTGs.
III. Radioisotope thermoelectric generators
All except one of the nuclear power packs listed in table 21.1 fall into this category. As their name implies, they consist of a radioisotope suitably packed and coupled to a system of parallel thermoelectric generators. The isotope which has become established for the purpose is plutonium-238.2 “This isotope has become the workhorse heat source for the majority of systems: past, present or future. Its 87.7-year half-life and its alpha emission decay scheme combine to enable design and development of space power supplies with light weight and long term operational reliability”.3 Figure 21.2 shows the decay scheme of plutonium-238. Regarding the trends towards increased electric power outputs and efficiencies of RTGs, the current goal of 1-2 kW(e) for flight test in 1982-83 seems, quite realizable and should obviate the need for further nuclear fission reactors in space in the near future.
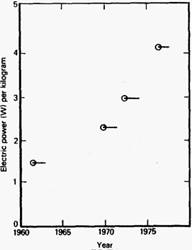
Note: The above figure shows the specific power in electrical watts output per kilogram of RTG mass for the US Snap and MHW devices. The dates are the first launchings into space3
IV. Re-entry of RTGs
The intact re-entry policy adhered to by the USA renders the re-entry of RTGs and reactors about as dangerous as the re-entry of a similar sized vehicle not containing radioactive material. The safety tests to which RTGs have been subjected are very comprehensive and the procedures are outlined in Use of Nuclear Power in Space by the United States of America.3 For arbitrary re-entry of a body of effective cross-sectional area A to a planetary surface of area AP in which the population of living beings L is NL, the probability of death or injury, P, is given by for small angles λ, where λ is the angle of inclination of the orbit to the equator, and where it is to be understood that the area A includes allowance for the size of the struck being and possible perimetric effects at the point of impact.1 For an effective area A of 12 square metres and a world population of 4 x 109 people, the chance of injury is about one per 10000 re-entries. In a future with a quadrupled world population and 1000 such re-entries per year, the number of expected deaths would still be less than one per annum on average, that is, too low to be recorded in typical statistical records, but nevertheless non-zero. These estimates assume that people are spread over the Earth’s surface, so that the probability of several people being struck by a single re-entering satellite is negligible. For an effective area 10 times larger, 120 square metres, the number of re-entries would have to be held to approximately 1,200 each year to keep accidents below 1 per annum out of a 16 x 109world population.
The number of people killed by lightning in Canada in 1975 and 1976 was 12 people each year, or about one per million per two years. Clearly the expected re-entry casualties are several orders of magnitude lower than this. The comparison with lightning is useful because lightning is not generally considered a major menace to life and limb. Too much attention to the intact re-entry problem could divert useful human energy from attention to much greater dangers which we face every day.
The comparison with lightning is useful because lightning is not generally considered a major menace to life and limb. Too much attention to the intact re-entry problem could divert useful human energy from attention to much greater dangers which we face every day.
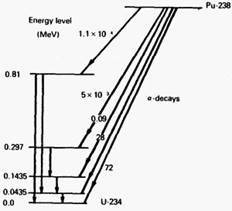
Note: The decay scheme of plutonium-238, showing the five strongest α-decay branches, and their percentages alongside the inclined arrows. All other α-decay branches are much weaker than 10-4 per cent and have been omitted for clarity. The α-particles will be entirely absorbed within the RTG, mainly within the plutonium itself, and thus give rise to the predominant source of heat. The gamma-ray decays which follow four of the five branches are shown by vertical arrows. The lower energy gammas will be strongly absorbed in the plutonium, and only the radiation from the 0.81 MeV level of U-234 will have an appreciable probability of escape. Since this radiation occurs only once in about 106decays, the radiation observed externally to a strong plutonium-238 source is relatively feeble.4
V. The Canadian experience with the Cosmos-954 satellite: operation ‘Morning Light’
The story of the search for the fallen pieces of this satellite which re-entered the atmosphere on 24 January 1978 is well known from many newspaper accounts. A map of the areas which were searched during the winter is given in figure 21.3 and details of items recovered are listed in table 21.2. The search began without delay and continued until 10 April. A second search for small radioactive particles has since been contracted out to industry by the Atomic Energy Control Board, because it was known from the winter search that some particles had drifted, in the course of their descent, far south of the main search zones.
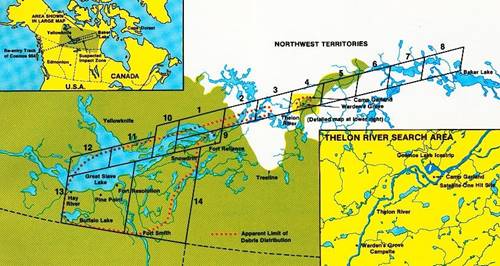
The Cosmos satellite was stated, through official channels of the Soviet Union, to contain an enriched uranium reactor. Some selected facts about the descent of the satellite and the search operation, Morning Light, are as follows:
- No part of the satellite struck any person directly as it fell.
- Nevertheless, two naturalists who were unaware that a satellite had fallen in their area found pieces of it sticking out of a crater in the Thelon River ice on 29 January. They are reported to have touched their find5 not realizing that it might be radioactive. No radioactive contamination was found on either of the two men in subsequent testing5.
- Several of the satellite pieces which were recovered were highly radioactive.
- One of the pieces was so highly radioactive that handling it without long tools could easily have been fatal. It required a special container to be made for its removal.
- It is very unlikely that all the pieces, especially the tiny specks of uranium, have been found; but the fission-fragment activity will have died down to a safer level by mid-1978. However, this very feature of reduced activity also makes the search for these particles more difficult.
- No particular human distress seems to have been caused by the Morning Light operation, but people unfamiliar with the general climate in the search area should bear in mind that temperatures of -40°C were normal, sometimes with high winds. There was also a possibility of losing a Hercules aircraft and crew on a runway created on a frozen lake, the ice having been of minimum thickness to bear a large aircraft.
- The total flying time for search aircraft of all types was 4634 hours as of 10 April 1978.
Table 21.2. Items recovered from the Cosmos-954 satellite
Part no. | location | Description no. | Dimensions (cm) | Radioactivity |
Search area one: east end of Great Slave Lake, near Fort Reliance NWT |
||||
1 | 62°50.5’N 109°29.5’W | Metal piece | 23 x 9 x 1.3 | Fission product |
2 | 62°52.1’N 109°32’W | Grey metal crushed ring black liner | 2 x 2 x 5 | Fission |
3 | 62°52’N 109°40′ W | Pipe, metals (Mn, Mg, Pb, Fe, Cu) and fibres | 25 dia x 30 long | |
4 | 62°57.3’N 109°S.5’W | Semi-circular metal plate | 2 x 2 x 8 | Radioactive |
5 | 62°54.3′ N 109°01’W | Thin metal,leaf-ribbed | 7 dia | Radioactive |
6 | 62°56.5′ N 100°S’W | Solid cylinder metal | 2 dia x 10 long | 25R/h at contact |
7 | 62°56’N 100°S.4′ W | Solid cylinder | 2 dia x 10 long | 30R/h at contact |
8 | 62°43’N 109°59’W | Oval, curved metal flake | longest dimension 5 | 10R/h at contact |
9 | 62°42’N 110°42’W | Solid cylinder metal | 2 dia x 10 long | 10R/h at contact |
10 | 62°53.5’N 109°26′ W | Solid cylinder | 2 dia x 9 long | 100mR/h at 1 m |
11 | 62°33’N 109°33’W | Three flakes | 100mR/h at 1 m | |
Search area two:vicinity of Artillery Lake, NWT |
||||
1 | 62°5S’N 105°57’W | Solid cylinder | 2 dia x 10 long | 5R/h at contact |
2 | 62°59′ N 105°55′ W | Solid cylinder | 10 long | 25R/h at contact |
3 | 63°0.5’N | Solid cylinder | 2 dia x 10 long | 100R/h at contact |
Search area four: near Warden’s Grove on Thelon River, NWT |
||||
1 | 63°47.8’N 104°13.5’W | Metal struts attached to soft metal plate | 3 long products | Activation |
Search area nine: South-east of Great Slave Lake, NWT |
||||
1 | 62°36′ N 110°46’W | 50 small pieces | each < I dia | Each 1-3R/h at contact |
Search area ten: South-east of Great Slave Lake, NWT |
||||
1 | 62°22’N 110°40’W | Solid black cube | I x I x I | 500R/h at contact |
Note: The list of parts found given in this table is incomplete: on 13 April the Canadian Department of External Affairs presented a diplomatic note (No. F1O-0840) to the embassy of the Soviet Union giving the total number of 10cm long by 2 cm diameter cylinders found as 29, while six cylinders 25 cm long by 9.5 cm diameter are also stated to have been found.
The 2 cm diameter cylinders are stated to be of beryllium. The larger cylinders appear also to be beryllium, possibly solid beryllium. A Soviet diplomatic note (No. 37) replying to the Canadian note gives the number of 2 cm diameter beryllium cylinders carried by the Cosmos954 satellite reactor as “several dozen” and states that most Qf them have been discovered. See also the map of the area in figure 21.3.
VI. Discussion and legal questions
The re-entry of Cosmos-954 and subsequent finding of small pieces reveals first of all that this type of spacecraft falls between the two extremes of intact re-entry and complete burn-up in the stratosphere. This intermediate category of partial burn-up seems to be the most inconvenient. The effective area A for collision of a falling satellite with a person or people would generally be larger than it would be for intact re-entry, and most certainly the hazards of radioactive contamination at ground level are relatively large. The harmlessness of the Cosmos-954 re-entry is generally attributed to the extremely sparse population of the winter wilderness it fell into, yet two naturalists found part of the debris by chance, not knowing at the time what it was. A definite risk of serious radiation sickness occurred despite the very low population density in the district. The first questions which may very naturally be raised are those concerning satellites which are already in Earth orbits and for which it is therefore too late to plan a different technology. There are the questions of: (a) warning of an impending reentry of a nuclear-powered satellite; (b) predicting and informing the state(s) where re-entry is likely to occur; (c) informing that re-entry has already occurred; (d) imparting of appropriate technical knowledge by the launching country to the states involved in post-re-entry search operations; and (e) supplying help for post-re-entry search operations. These first questions are all legal rather than technical, although same technical questions can emerge from them. None of these questions is necessarily adequately dealt with under the 1975 Convention on the Registration of Objects Launched into Outer Space, because of the special circumstances posed by the re-entry of radioactive materials. Accordingly a working paper6 by 14 member states of the UN Committee on Peaceful Uses Of Outer Space (UNCPUOS), dated 11 April 1978, called for a review by the Legal Subcommittee of UNCPUOS of these very questions, and also questions of notification before and at launching.
Questions regarding satellites which have not yet been launched can be both legal and highly technical. A change in planning procedures for design and use of satellites containing radioactive material could require international agreement through the United Nations, although it is possible that a change might be made unilaterally by a nation which launches such satellites. For example, such a change was made by the United States when intact reentry was decided upon. A change could also result from unprompted cooperation between nations.
It will also be useful to consider further developments at the United Nations and a range of questions about radioactive materials in space on which agreement should be reached.
On 13 February 1978 William H. Barton, Ambassador and Permanent Representative of Canada to the United Nations, issued a statement (Press Release No.2) to the Scientific and Technical Subcommittee (STS) of UNCPUOS. The statement was devoted entirely to the Cosmos-954 re-entry and considerations following from it. Referring to the STS he said, “The overall objective of our efforts should be to develop a regime for the use of nuclear power sources in outer space which would ensure the highest standards of safety for mankind and protection for the environment …” He proposed that a working group of technical and scientific experts be formed to carry out a detailed review and technical study for the STS, to prepare the ground for constructive action in the legal Subcommittee of UNCPUOS, and in the UNCPUOS itself and the General Assembly. A decision to form such a working group was reached in the June-July session of UNCPUOS in New York.
Many of the questions which should be discussed in the working group have already been raised in a working paper7. Others, perhaps, may not yet have been considered. In what follows, a range of questions will be mentioned: (a) available power sources for satellites: their relative advantages and disadvantages; (b) radiation standards for re-entering satellites; (c) restrictions on the orbits of satellites which would supplement and reinforce (b); (d) design standards and tests which would ensure either intact reentry on the one hand, or complete dispersal in the stratosphere on the other hand; (e) consideration of equipping intact re-entry satellites with rocket motors which could be activated years after satellite launching, so as to make it possible for re-entry to occur at a chosen location; and (f) legal inclusion of nuclear reactors and RTGs in satellites within the category of devices for which open exchange of information already exists. The first four questions (a) to (d), above, are already included in the proposed agenda7 of the working group of the STS. Questions (e) and (f) appear to to have been formally proposed.
Question (e) refers only to intact re-entry and should be considered if intact re-entry is decided upon, especially the intact re-entry of reactors which could in principle go critical, for example, in the ocean. This suggestion poses problems which may be technically severe especially when the reentry date is many years after launch. Nevertheless, this question should be investigated and could result in a strong preference for RTGs being launched in preference to nuclear reactors. The more restricted question of the control of the position of re-entry of an RTG is less sensitive because, as shown above, an intact re-entering RTG is unlikely to hurt anyone. The occurrence of uncontrolled RTG re-entry could therefore be felt to be one that could be classified legally as an ‘inevitable accident’. Question (f) has interesting ramifications in nuclear cooperation, technological cooperation (for example, over thermoelectric generators), and even offers opportunities for international gestures of a tension-reducing nature. At one extreme one can even foresee the envisaged technological exchanges being coupled to moves in disarmament. Such exchanges may be relevant to the future design of surveillance satellites and would thus be in the interest of world security. For these and other reasons the technology of powering satellites should no longer be held in the realm of military secrets.
VII. Other power sources for satellites and the trend towards higher electrical power output
For space missions to remote parts of the solar system, far outside the radius of the Earth’s orbit, there may be no suitable choice of power system other than reactors or RTGs. For Earth orbits, however, the use of solar cells is very well established. The practical efficiency of energy conversion using solar cells is furthermore fast approaching a value of 15 per cent8,9 which, combined with the solar constant of 1.38kW/m-2 at the position of the Earth, leads to the conclusion that solar panels in space may soon yield 100W(e)/m2. The limits to the power output would be dictated by the maximum mass which one could afford to launch, for example, as dictated by the maximum mass for a single shuttle.
The comparative limitations of various systems are illustrated in figure 21.4, which is intended to apply to missions contemplated by the US Department of Defense for geosynchronous orbits. The solar energy power boundary is seen to flatten at the level of 40 to 50 kW(e), presumably because some batteries must be carried aboard the power unit to take care of occultation. It is the mass of the batteries, even using the most favourable projections9 from work on new types of battery, which sets a low ceiling on the power which can be expected to be brought into orbit in a single shuttle. The solar power capability could increase by more than a factor of three where batteries are not required.
Also the limitation of RTGs in figure 21.4 is not purely technical but represents the design limits of RTGs currently being developed for test and space missions in the early 1980s. Doubtless it also reflects to some extent the high cost of plutonium-238, which was $650 per thermal watt for the unencapsulated isotope (in 1973 prices). If only energy possibilities are considered, at 20 kW(e) the piutonium-238 mass could be held to roughly 200 kg, given thermoelectric converters of the improved efficiencies now being developed, so that the mass of the RTG could be kept within the rule of thumb value10 of 955 kg for a single shuttle. Clearly, somewhere between 20 kW(e) and 200 kW(e) the RTG ceases to be practical by comparison with the nuclear reactor. The problems of cooling reactors and RTGs in space are similar. The assembly and launch problems with RTGs are likely to be worse than with reactors, since the latter are launched before start-up, whereas the plutonium-238 is always hot. thermally. Although the radioactivity is mainly self-contained, the rarer radioactive branch decays of the radioisotope (figure 21.2) will add to the hazards of launching very active RTGs.
Finally, the RTG offers the only sound prospect of a reliable power source having a lifetime considerably in excess of the seven to ten years indicated at the right in figure 21.4. Solar cells will deteriorate over periods of years due to radiation damage, and reactors would require refuelling.
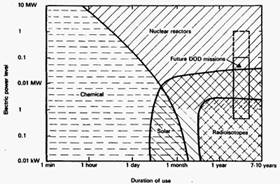
Concluding remarks
This paper has been based mainly on the knowledge which is available of the current achievements in the production of electric power for space applications using nuclear sources, and on the foreseeable developments into the early 1980s. This could be too narrow a basis for an international agreement which is to be of reasonably long range. Therefore the more distant future prospects have been touched upon by making a few comments about figure 21.4, so as to begin to examine some of the questions which will be faced if much greater electric power is required in future space missions. To do justice to these very complex problems, it would be necessary to assemble the separate views of technical experts in each of the fields of reactor, RTG and solar power system design. It would not appear that reactors offer distinct advantages over RTGs up to power levels of at least 20 kW(e), apart from the high cost of the isotope plutonium-238. For satellites intended to have a powered lifetime much longer than seven years, reactors and solar cells may not be practical, and the RTG may be the only choice. Solar cell arrays for space use are moving towards a power-tomass ratio of 200 W(e)/kg; already power outputs of over 150 kW(e) (single shuttle) seem realistic for a satellite which does not have to carry batteries. However, most systems to date have required electrical storage, so that power is maintained during occultation. Even projecting to the most advanced future batteries, this would reduce the solar power system output to about 40 kW(e) for the same mass.
Acknowledgements
I would like to thank the following people for help in finding information or for sending material on which this article is based: Dr R. J. Drachman and Dr Jerome Mullin of NASA; Mr B. J. Rock of the US Department of Energy; Dr D. Buden and Dr C R. Emigh of the Los Alamos
Scientific Laboratory; Dr E. S. Rittner of the Comsat Laboratories; Capt. S. B. Burton of Canada’s Department of National Defence; Messrs Hugh Spence and G. B. Knight of the Atomic Energy Control Board; and lastly Messrs Erik Wang and P. McRae of the Department of External Affairs. Personal thanks are also due to Dr K. Jung and to A. L. who made useful comments on earlier versions of this paper.
References
- UN document A/AC.105/PV.187. [↩] [↩]
- Buden, D., Nuclear Reactors/or Space Electric Power, Report No. LA-7290-SR, UC-33 and UC-80, Los Alamos, June 1978. [↩] [↩]
- Use of Nuclear Power in Space by the United States of America (Department of Energy, Washington, D.C, 1978). [↩] [↩] [↩] [↩]
- Lederer, C. M., Hollander, J. M. and Perlman, I., Table of Isotopes (J. Wiley, New York, 1968). [↩]
- Operation Morning Light, General information fact sheet, Department of National Defence (Ottawa, April 1978). [↩] [↩]
- UN document A/ACI05/C.2ILlI5 [↩]
- UN document A/AC.I05/C.I/L.103. [↩] [↩]
- Rittner, E. S., ‘Recent advances in components of space power systems’, Proceedings of the 25th International AstronaUtical Congress (Pergamon, Oxford, 1976), PI’. 137-46. [↩]
- Goldsmith, P. and Reppucci, G. M., ‘Advanced photovoltaic synchronous orbit spacecraft power systems’, Journal of Energy, Vol. 2, No. 81 (1978). [↩] [↩]
- Reactors for Space Electrical Power, ERDA Contract No. W7405-ENG. 36, Los Alamos Scientific Laboratory, January 1977. [↩]